We are surrounded by waves. tiny vibrational waves transport sound to our ears. Light waves stimulate the retinas of our eyes. Electromagnetic waves bring radio, television and endless streaming content to our devices. Remarkably, all these different waves are governed largely by the same fundamental physical principles. And recently there has been a revolution in our ability to control these waves using materials, engineered at the nanoscale, known as metamaterials.
The Greek prefix meta means “beyond.” These engineered materials let us move beyond the traditional ways in which waves and matter interact, creating technologies where light and sound appear to disobey conventional rules. The marquee example of this new style of materials is the “invisibility cloak”—a metamaterial coating that can hide an object in plain sight. Several research teams around the world, including mine, have designed and produced metamaterial coatings that can redirect light waves that hit them, effectively preventing light from bouncing off the object and reaching our eyes and even from leaving shadows. Although these inventions have limitations—they aren't quite the Harry Potter–style invisibility cloaks that many people imagine—they nonetheless interact with light in a way that seems like magic.
Cloaks are just one example of metamaterial technology. Other metamaterials allow light to travel one way but not the opposite—a valuable tool for communication and detection of objects—and to break symmetries of geometry and time. With modern nanofabrication tools and a better understanding of how light and matter interact, we can now structure metasurfaces to produce any pattern, color and optical feature we can think of.
On supporting science journalism
If you're enjoying this article, consider supporting our award-winning journalism by subscribing. By purchasing a subscription you are helping to ensure the future of impactful stories about the discoveries and ideas shaping our world today.
Bending and Twisting Light
For centuries scientists have strived to control the properties of light and sound as they interact with our sensory systems. An early success in this quest was the invention of stained glass: ancient Romans and Egyptians learned how to melt metallic salts into glass to tint it. The tiny metal nanoparticles dispersed in the glass absorb specific wavelengths and let others through, creating bright colors in masterpieces that we still admire today. In the 17th century Isaac Newton and Robert Hooke recognized that the hue and iridescence of some animals are created by nanoscale patterns on the surface of their body parts—another example of how nanostructured materials can create surprising optical effects.
Human eyes are excellent at detecting two fundamental properties of light: its intensity (brightness) and its wavelength—that is, its color. A third important property of light is its polarization, which describes the trajectory that light's electromagnetic fields trace in space over time. Although humans cannot distinguish one polarization from another with our eyes, several animal species have polarization sensitivity, allowing them to see more, better orient themselves in their surroundings and signal to other creatures.
In the late 19th century, a few years after James Clerk Maxwell's discovery of the equations of electromagnetism, Jagadish Chandra Bose built the first examples of what we could call a metamaterial. By manually twisting jute fibers and arranging them in regular arrays, he demonstrated that linearly polarized electromagnetic waves—light whose electric and magnetic fields oscillate along straight lines—rotate their polarization as they propagate through and interact with the jute structures. Bose's twisted jute showed that it was possible to engineer an artificial material to control light in unprecedented ways.
The modern era of metamaterials can be traced back to 2000, when physicists David R. Smith of Duke University, the late Sheldon Schultz of the University of California, San Diego, and their colleagues created an engineered material unlike any seen before—a material with a negative index of refraction. When a beam of light travels from one medium to another—from air to glass, say—its speed changes, causing the beam to bend, or “refract.” The difference in index of refraction between the two materials defines the angle of that bending.
Refraction phenomena are the basis of most modern optical devices, including lenses and displays, and explain why a straw in a glass of water looks broken. For all known natural materials, the index of refraction is positive, meaning that light always bends on the same side of the interface, with a larger or smaller angle from the interface as a function of the change in index. Light entering a medium with a negative index of refraction, on the contrary, would bend backward, creating unexpected optical effects, such as a straw appearing to lean the wrong way. Scientists long assumed that it was impossible to find or create a material supporting negative refraction, and some argued that it would violate fundamental physical principles. When Schultz, Smith and their colleagues combined tiny copper rings and wires on stacked circuit-board substrates, however, they demonstrated that a microwave beam passing through this engineered material undergoes negative refraction. This striking advance showed that metamaterials can yield a much wider set of refractive indexes than nature offers, opening the door to totally new technological possibilities. Since then, researchers have created negative-index materials for a wide range of frequencies, including for visible light.
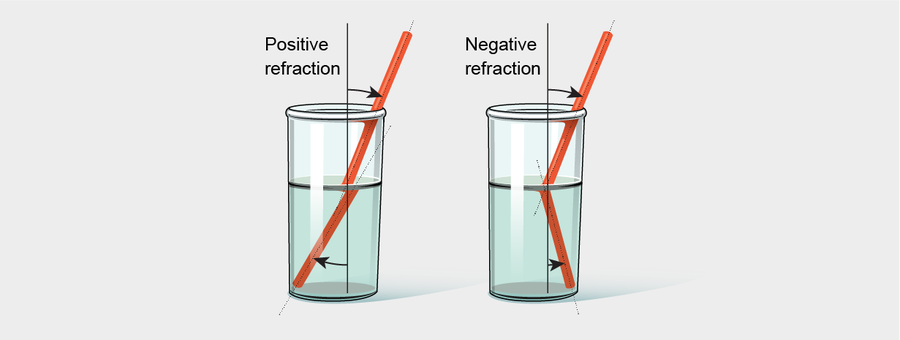
Credit: Jen Christiansen
Cloaking Technologies
After this initial breakthrough, a great deal of metamaterial research focused on cloaking. Nearly 20 years ago, while I was working with Nader Engheta of the University of Pennsylvania, we designed a metamaterial shell that would make an object undetectable by causing the light waves bouncing off the shell to cancel out the light waves scattered from the cloaked object. No matter which direction it came from, a wave that hit the structure would be redirected by the cloak in a way that canceled the wave scattered by the object itself. As a result, the cloaked object would be impossible to detect via external illumination: from an electromagnetic point of view, it would appear not to exist.
Around the same time, John B. Pendry of Imperial College London and Ulf Leonhardt, now at the Weizmann Institute of Science in Rehovot, Israel, proposed other interesting ways to use metamaterials to cloak objects. And within a few years various experimental demonstrations turned these proposals into reality. My group, for instance, produced a three-dimensional cloak that can drastically reduce the amount of radio waves that scatter off of a cylinder, making it difficult to detect via radar. Existing stealth technologies can hide objects from radar by absorbing the impinging waves, but metamaterial cloaks do much better because they don't just suppress the reflected waves—they reroute the incoming waves to eliminate scattering and shadows, making the cloaked object undetectable. We and other groups have extended cloaking to acoustic (sound) waves, creating objects that can't be detected by sonar devices. Other scientists have even made cloaks for thermal and seismic waves.
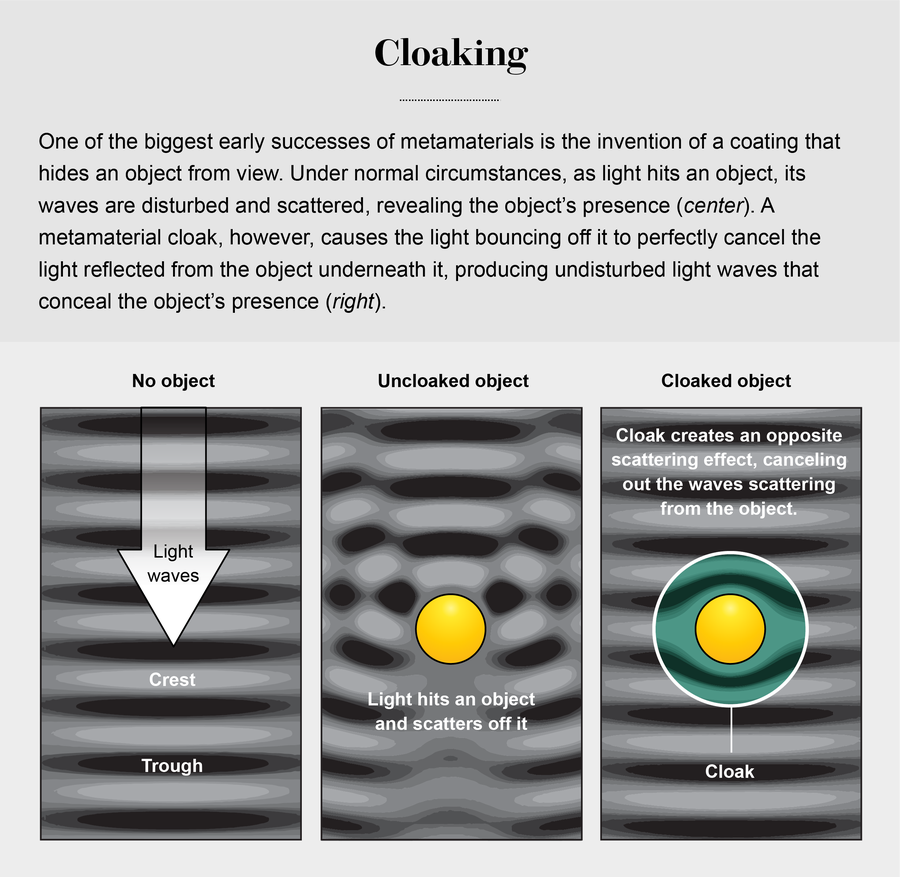
Credit: Jen Christiansen
There is, however, a long way to go from these devices to invisibility cloaks like those pictured in movies, which allow the multiwavelength background behind an object to shine through. Our real-life cloaks are limited to either small sizes or narrow wavelengths of operation. The underlying challenge is the competition against the principle of causality: no information can travel faster than the speed of light in free space. It is impossible to fully restore the background electromagnetic fields as if they were traveling through the object without slowing them down.
Based on these principles, my group has demonstrated that we cannot completely suppress scattering from an object at more than a single wavelength (a single color of light) using a passive metamaterial coating. Even if we induce only partial transparency, we face a severe trade-off between how big the cloaked object can be and how many colors of light we can cloak it for. Cloaking a large object at visible wavelengths remains far-fetched, but we can use metamaterial cloaks for smaller objects and longer wavelengths, with exciting opportunities for radar, wireless communications and high-fidelity sensors that don't perturb their surroundings as they are operated. Cloaks for other kinds of waves, such as sound, have fewer limitations because these waves travel at much slower speeds.
Spatial Symmetries
A particularly powerful tool for designing and applying metamaterials for various purposes is the concept of symmetry. Symmetries describe aspects of an object that do not change when it is flipped, rotated or otherwise transformed. They play a fundamental role in all natural phenomena. According to a 1915 theorem by mathematician Emmy Noether, any symmetry in a physical system leads to a conservation law. One example is the connection between temporal symmetry and energy conservation: if a physical system is described by laws that do not explicitly depend on time, its total energy must be preserved. Similarly, systems obeying spatial symmetries, such as periodic crystals that remain the same under translations or rotations, preserve some properties of light, such as its polarization. By breaking symmetries in controlled ways, we can design metamaterials to overcome and locally tailor these conservation laws, enabling novel forms of light control and transformation.
As an example of the powerful role of symmetries for metamaterial design, my group has engineered an optical metamaterial that can efficiently rotate the polarization of light that travels through it—in some ways, it is a nanoscale version of Bose's twisted jute arrangement. The material is made of multiple thin layers of glass, each one embedded with rows of gold rods, tens of nanometers long. First, we create one layer of nanorods all oriented in a certain direction over the glass. Then we stack a second layer, which looks identical to the first, except that we rotate all the rods at a specific angle. The next layer is adorned by nanorods further rotated by this same angle, and so on. Overall, the stack is only about a micron thick, yet it features a specific degree of broken spatial symmetry compared with natural periodic crystals, where molecules are all lined up in straight rows. As light passes through this thin metamaterial, it interacts with the gold nanorods and is slowed down by electron oscillations at their surface. The emerging light-matter interactions are controlled by the twisted symmetry of the crystal lattice, enabling a large rotation of the incoming light polarization over a broad range of wavelengths. This form of polarization control can benefit many technologies, such as liquid-crystal displays and sensing tools used in the pharmaceutical industry, which rely on polarization rotation that typically emerges much less efficiently in natural materials.
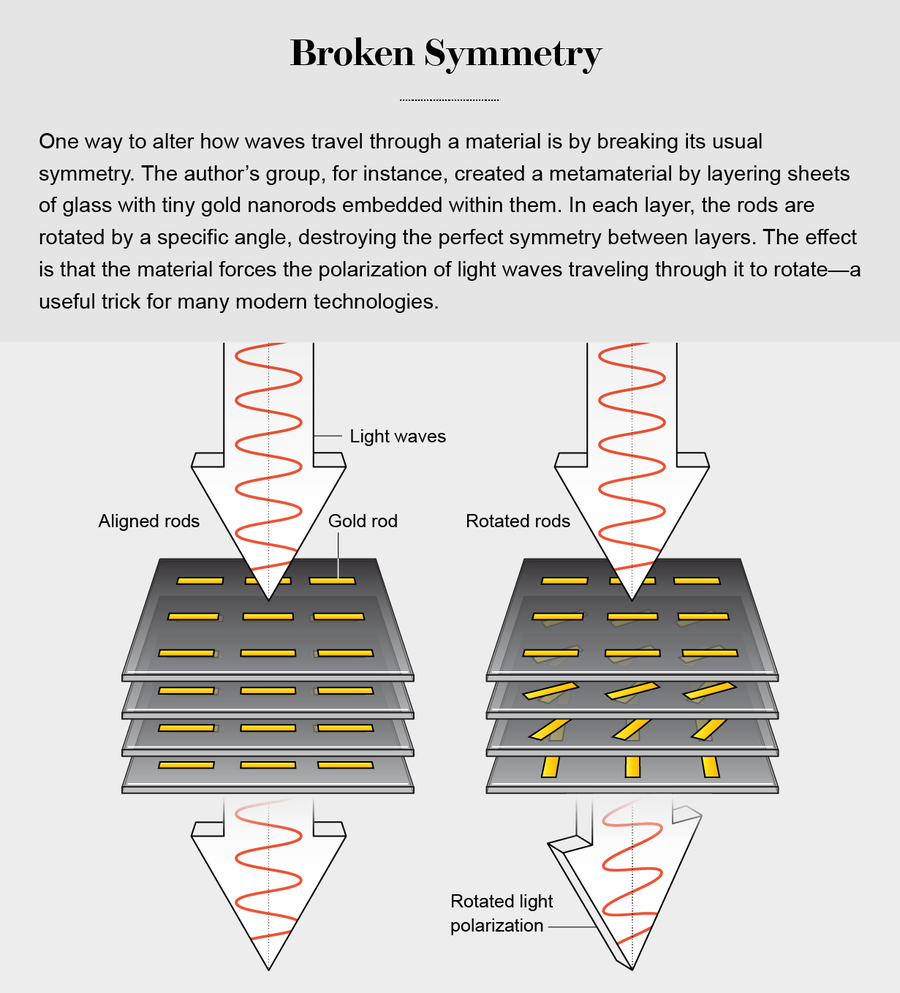
Credit: Jen Christiansen
Underlying rotational symmetries also play a crucial role in governing other metamaterial responses. Pablo Jarillo-Herrero's group at the Massachusetts Institute of Technology recently showed that two closely spaced layers of graphene—just a single layer of atoms each—carefully rotated with respect to one another by a precise angle result in the striking emergence of superconductivity. This feature, which the two layers individually do not possess, allows electrons to flow along the material with zero resistance, all because of the broken symmetry induced by the twist. For a specific rotation angle, the emerging interactions between the neighboring atoms in the two layers define a totally new electronic response.
Inspired by this demonstration, in 2020 my group showed that a somewhat analogous phenomenon can occur not for electrons but for light. We used a stack of two thin layers of molybdenum trioxide (MoO3) and rotated one with respect to the other. Individually each layer is a periodic crystal lattice, in which the underlying molecules are arranged in a repeating pattern. When light enters this material, it can excite the molecules, causing them to vibrate. Certain wavelengths of light, when polarized in a direction aligned with the molecules, prompt strong lattice vibrations—a phenomenon called a phonon resonance. Yet light with the same wavelength and perpendicular polarization produces a much weaker material response because it does not drive these vibrations. We can take advantage of this strong asymmetry in the optical response by rotating one layer with respect to the second one. The twist angle once again controls and modifies the optical response of the bilayer in dramatic ways, making it very different from that of a single layer.
For example, light emitted by a molecule placed on the surface of a conventional material such as glass or silver flows outward in circular ripples, as when a stone hits the surface of a pond. But when our two MoO3 layers are stacked on top of each other, changing the twist angle can drastically alter the optical response. For a specific twist angle between the crystal lattices, light is forced to travel in just one specific direction, without expanding in circular ripples—the analogue of superconductivity for photons. This phenomenon opens the possibility of creating nanoscale images beyond the resolution limits of conventional optical systems because it can transport the subwavelength details of an image without distortion, efficiently guiding light beyond the limits imposed by diffraction. Light in these materials is so strongly linked to material vibrations that the two form a single quasiparticle—a polariton—in which light and matter are strongly intertwined, offering an exciting platform for quantum technologies.
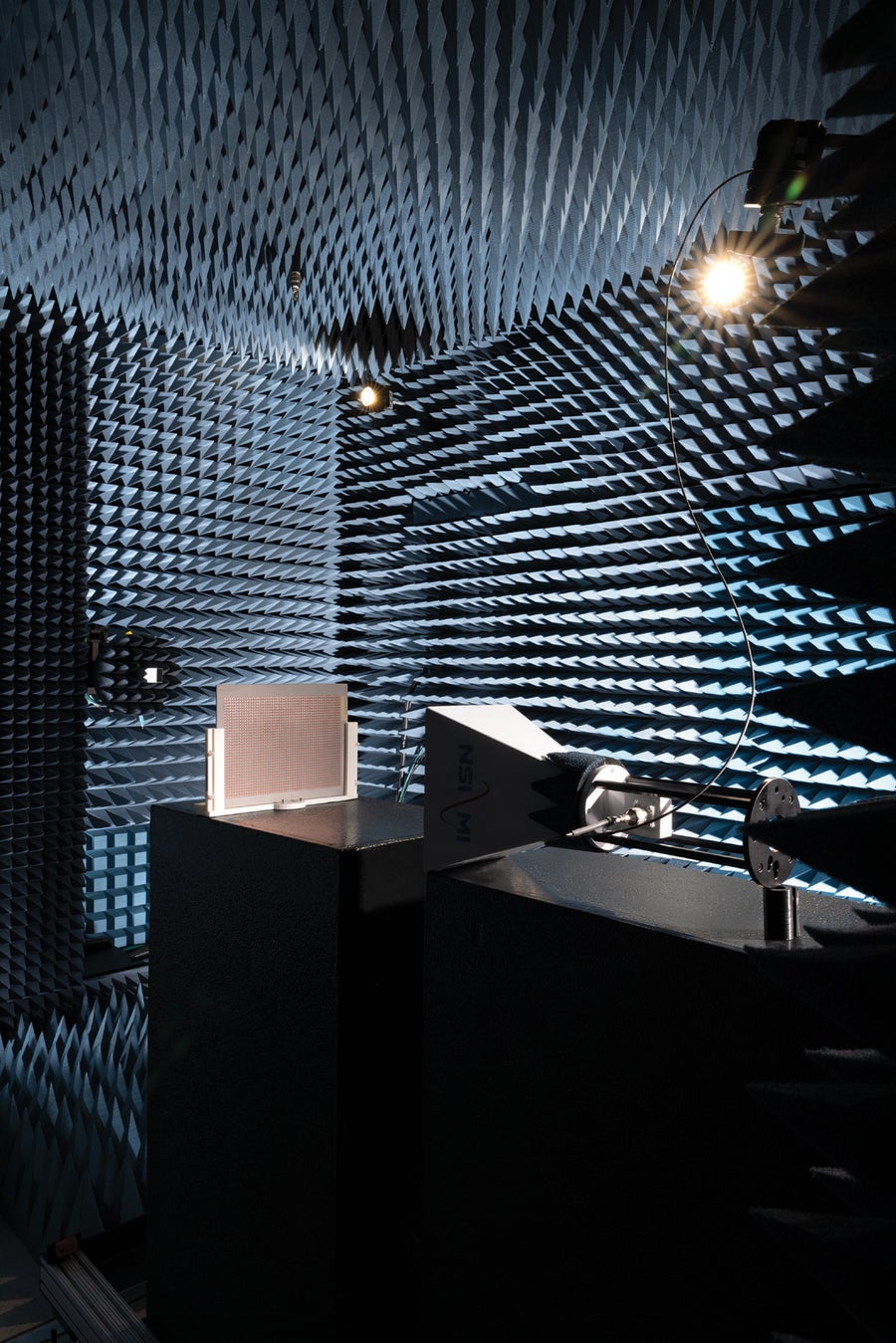
A metamaterial undergoes testing in a chamber that enables very precise measurements of radio and millimeter-wavelength light. Credit: Craig Cutler
Symmetries in Time
The role of symmetry in metamaterials is not limited to spatial symmetries, such as the kind broken by geometric rotations. Things get even more interesting when we experiment with breaking time-reversal symmetry.
The equations that govern wave phenomena are typically reversible in time: if a wave can travel from point A to point B, it can also travel back from B to A with the same features. Time-reversal symmetry explains the common expectation that if we can hear or see someone, they can also hear or see us. Breaking this symmetry in wave transmission—known as reciprocity—can be important for many applications. Nonreciprocal transmission of radio waves, for instance, can enable more efficient wireless communications in which signals can be transmitted and received at the same time without interference, and it can prevent contamination by the reflection of signals you send out. Nonreciprocity for light can protect sensitive laser-beam sources from unwanted reflections and provides the same benefit in radar and lidar technologies.
An established way to break this fundamental symmetry exploits magnetic phenomena. When a ferrite—a nonmetallic material with magnetic properties—is subject to a constant magnetic field, its molecules sustain tiny circulating currents that rotate with a handedness determined by the magnetic field orientation. In turn, these microscopic currents induce a phenomenon called Zeeman splitting: light waves with right-handed circular polarization (an electric field that rotates clockwise) interact with these molecules with a different energy than left-handed (counterclockwise) waves. The difference in energy is proportional to the applied magnetic field. When a linearly polarized wave travels through a magnetized ferrite, the overall effect is to rotate the polarization, in some ways similar to the metamaterials discussed earlier. The fundamental difference is that here the handedness of the polarization rotation is determined by the external magnetic bias, not by the broken symmetry in the metamaterial constituents. Hence, in these magnetized materials, light's polarization rotation has the same handedness when it's traveling in one direction as it does when it's moving in the opposite direction—a feature that violates reciprocity. Time-reversal symmetry is now broken.
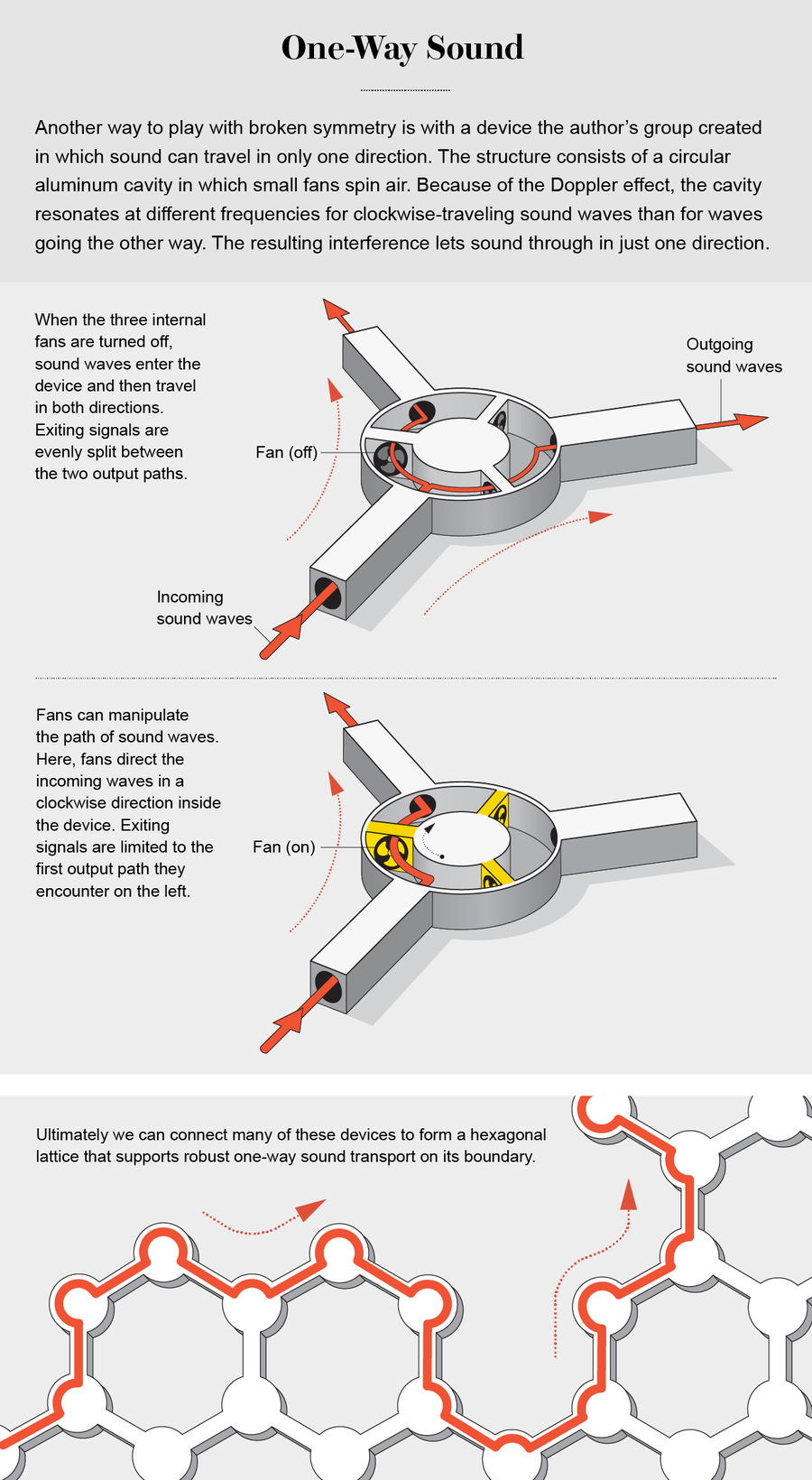
Credit: Jen Christiansen
We can exploit this phenomenon to engineer devices that allow waves to propagate in only one direction. Yet few natural materials possess the desired magnetic properties to achieve this effect, and those that do can be difficult to integrate into modern devices and technologies based on silicon. In recent years the metamaterials community has been working hard to create more efficient ways to break wave reciprocity without magnetic materials.
My group has shown that we can replace the tiny circulating currents in a magnetized ferrite with mechanically rotating elements in a metamaterial. We achieved this effect in a single compact acoustic device by using small computer fans spinning air inside a circular aluminum cavity, creating a first-of-its-kind nonreciprocal device for sound. When we turn on the fans, the frequencies at which the cavity resonates are different for counterrotating sound waves, similar to how Zeeman splitting changes the energy of light's interactions in a ferrite. As a result, a sound wave in this rotating cavity experiences a very different interaction depending on whether it travels clockwise or counterclockwise inside it.
We can then route sound waves nonreciprocally—one way only—through the device. Remarkably, the airflow speed necessary to create this effect is hundreds of times slower than the speed of the sound waves, making this technology quite simple to develop. Such compact nonreciprocal devices can then form the basis of a metamaterial, made by connecting these elements in a lattice. These engineered crystal lattices transport sound in highly unusual, nonreciprocal ways, reminiscent of how electrons travel with unique features in topological insulators.
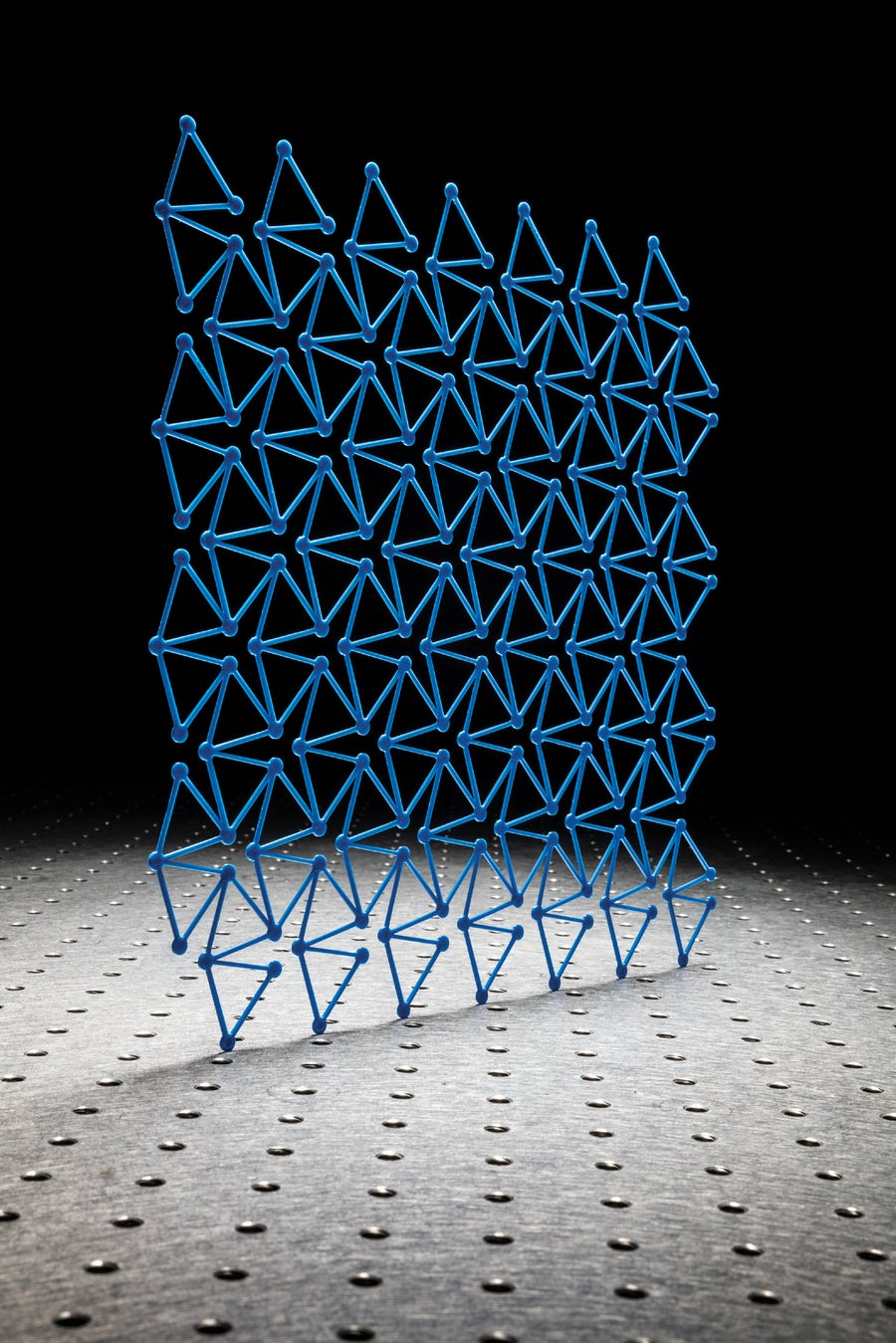
A metasurface for elastic waves can endow sound with highly unusual features. Tiny magnets at the corners of the triangles control the shape of the metasurface, dramatically varying its acoustic properties. Credit: Craig Cutler
Can we use a similar trick for light? In 2018 Tal Carmon's group at Tel Aviv University demonstrated an analogous effect by spinning the read-head of a hard-disk drive coupled to an optical fiber at kilohertz frequencies, demonstrating nonreciprocal transmission of light through it. The researchers' setup showed that mechanically rotating elements can be used to force light to travel through a device in one direction only. An arguably more practical route is to use metamaterials made of time-varying constituents that are switched on and off with specific patterns in space, mimicking rotation. Based on these principles, my group has created several technologies that operate efficiently as nonreciprocal devices. Their small footprints allow us to easily integrate them into larger electronic systems.
We have also extended these techniques to thermal emission, the radiation of light driven by heat. All hot bodies emit light, and a universal principle known as Kirchhoff's law of thermal radiation states that reciprocal materials in equilibrium must absorb and emit thermal radiation at the same rate. This symmetry introduces several limitations for device designs for thermal energy management and for energy-harvesting devices such as solar cells. By employing design principles similar to the ones described earlier to break light reciprocity, we are envisioning systems that do not obey the symmetry between absorption and emission. We can structure metamaterials to efficiently absorb heat without needing to reemit a portion of the absorbed energy toward the source, as a normal material would, enhancing the amount of energy we can harvest. Applied to static mechanics, analogous principles have also allowed us to create a 3-D-printed object that asymmetrically transmits an applied static mechanical force—a kind of one-way glove that can apply pressure without feeling the back action.
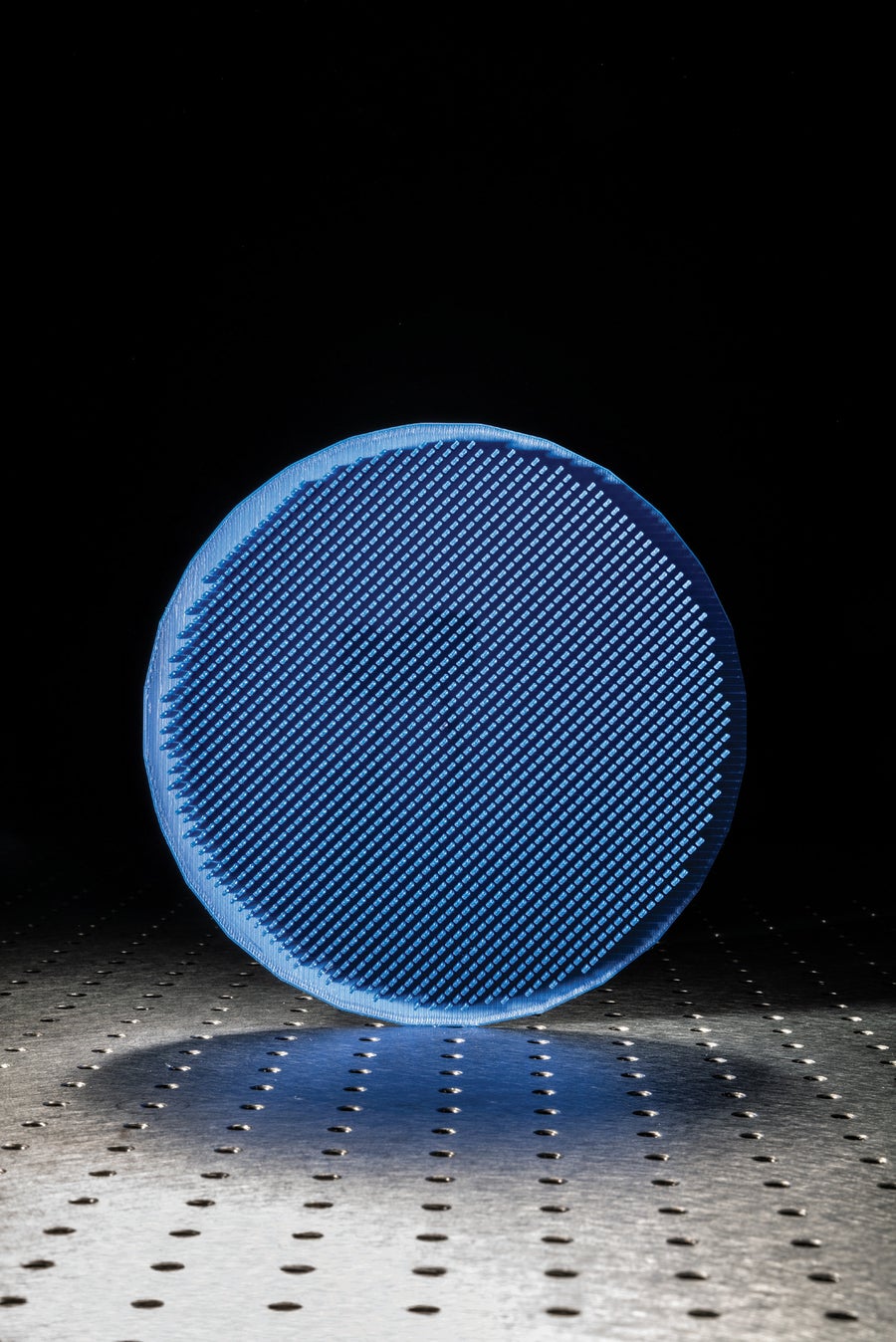
Mechanical vibrations propagate over a metasurface that can direct sound and strongly enhance its interactions with matter. Credit: Craig Cutler
Many More Wonders
The opportunities offered by metamaterials and broken symmetries to manipulate and control waves do not end here. Scientists have been discovering new ways to trick light and sound—for instance, by combining broken geometric symmetries and temporal symmetries in novel ways. Metamaterials can be featured on the walls and windows of smart buildings to control and route electromagnetic waves at will. Nanostructured metasurfaces can shrink bulky optical setups into devices thinner than a human hair, enhancing imaging, sensing and energy-harvesting technologies. Acoustic and mechanical metamaterials can route and control sound with an unprecedented degree of control. We expect many more wonders, given the enormous opportunities that modern nanofabrication techniques, our improved understanding of light-matter interactions, and sophisticated materials science and engineering present us.