We are often told that there are no shortcuts in life. But the brain—even the brain of a rat—is wired in a way that completely ignores this kind of advice. The organ, in fact, epitomizes a shortcut-finding machine.
The first indication that the brain has a knack for finding alternative routes was described in 1948 by Edward Tolman of the University of California, Berkeley. Tolman performed a curious experiment in which a hungry rat ran across an unpainted circular table into a dark, narrow corridor. The rat turned left, then right, and then took another right and scurried to the far end of a well-lit narrow strip, where, finally, a cup of food awaited it. There were no choices to be made. The rat had to follow the one available winding path, and so it did, time and time again, for four days.
On the fifth day, as the rat once again ran straight across the table into the corridor, it hit a wall—the path was blocked. The animal went back to the table and started looking for alternatives. Overnight, the circular table had turned into a sunburst arena. Instead of one track, there were now 18 radial paths to explore, all branching off from the sides of the table. After venturing out a few inches on a few different paths, the rat finally chose to run all the way down path number six, the one leading directly to the food.
On supporting science journalism
If you're enjoying this article, consider supporting our award-winning journalism by subscribing. By purchasing a subscription you are helping to ensure the future of impactful stories about the discoveries and ideas shaping our world today.
Taking the path straight to the food cup without prior experience may seem trivial, but from the perspective of behavioral psychologists at the time, the rat's navigational accomplishment was a remarkable feat. The main school of animal learning in that era believed that maze behavior in a rat is a matter of simple stimulus-response associations. When stimuli in the environment reliably produce a successful response, neural connections that represent this association get strengthened.
In this view, the brain operates like a telephone switchboard that maintains only reliable connections between incoming calls from our sense organs and outgoing messages to the muscles. But the behavioral switchboard was unable to explain the ability to correctly choose a shortcut right off the bat without having first experienced that specific path. Shortcuts and many other intriguing observations along these lines lent support to a rival school of thought promulgated by theorists who believe that in the course of learning, a map gets established in a rat's brain. Tolman—a proponent of that school—coined the term: the cognitive map.
According to Tolman, the brain does more than just learn the direct associations among stimuli. Indeed, such associations are often brittle, rendered outdated by changes in the environment. As psychologists have learned in the decades since Tolman's work, the brain also builds, stores and uses mental maps. These models of the world enable us to navigate our surroundings, despite complex, changing environments—affording the flexibility to use shortcuts or detours as needed. The hungry rat in Tolman's experiment must have remembered the location of the food, inferred the angle to it and chosen the route most likely to bring it to its goal. Quite simply, it must have built a model of the environment.
Such model building or mapmaking extends to more than physical space. Mental maps may exist at the core of many of our most “human” capacities, including memory, imagination, inferences, abstract reasoning and even the dynamics of social interactions. Researchers have begun to explore whether mental maps document how close or distant one individual is to another and where that individual resides in a group's social hierarchy. How does the brain, in fact, create the maps that allow us to make our way about the world?
A Spatial Map
The first hints of a neural basis for mental maps came in the 1970s. While studying a brain region called the hippocampus in rodents, John O'Keefe of University College London, along with his student Jonathan Dostrovsky, discovered a particular class of neurons that becomes active when mice occupy specific locations in their environment. Some of these neurons fired when the animal was in one location, and others switched on when it moved to the next spot on the path along which it traveled, as if the cells were specialized to track where the animal was in space. By linking sequences of these “place cells” together, researchers were able to reconstruct an animal's navigational trajectory. Work over the intervening decades confirmed the existence of place cells in other animals, including humans, and clarified many of their properties. Along the way, a host of cell types surfaced, each uniquely contributing to the brain's encoding of spatial representations.
In the nearby entorhinal cortex, a region connected to the hippocampus, a research team led by Edvard Moser and May-Britt Moser, former postdoctoral visiting fellows in O'Keefe's laboratory, discovered neurons highly similar to place cells. These cells also fired when an animal was in specific locations. But unlike place cells, each of these newly discovered cells spiked in multiple, regular locations. When mapped onto the animal's position, the activity patterns of these “grid cells” resembled highly regular, equilateral triangles. Like a spatial metric, these cells fired when an animal passed over the vertices of the triangles. The discovery of these cell types sparked excitement because of the emerging picture of how the brain controls navigation. Place cells and grid cells could provide a means to locate oneself in space and determine distance and direction. These navigational tools are crucial for building mental maps. (O'Keefe and the Mosers received the 2014 Nobel Prize in Medicine or Physiology for their work on place and grid cells.)
A wide variety of information is useful for creating such a map, and the hippocampus-entorhinal system encodes much of it. Discovering the location of a physical goal is one example: as an animal navigates toward an objective, some hippocampal neurons fire depending on the direction and distance to reach it. The cells increase their firing rate as the animal approaches the goal.
Other cells also enter the picture. A dedicated population of “reward” cells encodes reward locations across different environments, providing a signal to guide an animal's navigation (think of an “X” marking the spot of treasure on a pirate's map). Other cells track speed and direction and in doing so act like internal speedometers and compasses that compute an animal's progress as it travels through the environment. Specific cells that signal the locations of landmarks in the surroundings serve as references to correct errors in the animal's trajectory. A map must also have edges: cells that fire more as the animal approaches the map's perimeter.
For humans, the importance of such an abundance of cell types seems obvious: the brain is responsible for knowing the location of home and work, walls and dead ends, a favorite shop or the corner store. It is still a mystery as to how all of this information is drawn together into a coherent map, but these cells appear to provide the parts list for the elements of neural mapmaking.
This hippocampal-entorhinal system is more than a mapmaker, though, and the maps are more than a way to locate oneself in space. These maps also are used for active planning. When a rat comes to a junction in a familiar maze, it will pause while place cell firing sequences that relate to the different options are activated, as if the animal is contemplating the choices.
Humans engage similar processes. Research in participants navigating virtual environments while their brains were scanned with functional magnetic resonance imaging shows that the hippocampus becomes active in ways consistent with spatial planning, such as considering and planning routes.
Shaping of plans also occurs during sleep. Sequences of place cell activity can be reactivated during sleep to replay the past or simulate the future. Without the ability to simulate new behaviors, we would have to explore a multitude of real-world options before deciding on what action to take. We would be constant empiricists, able to act only on direct observations. Instead off-line simulations give us the ability to envision possibilities without directly experiencing them.
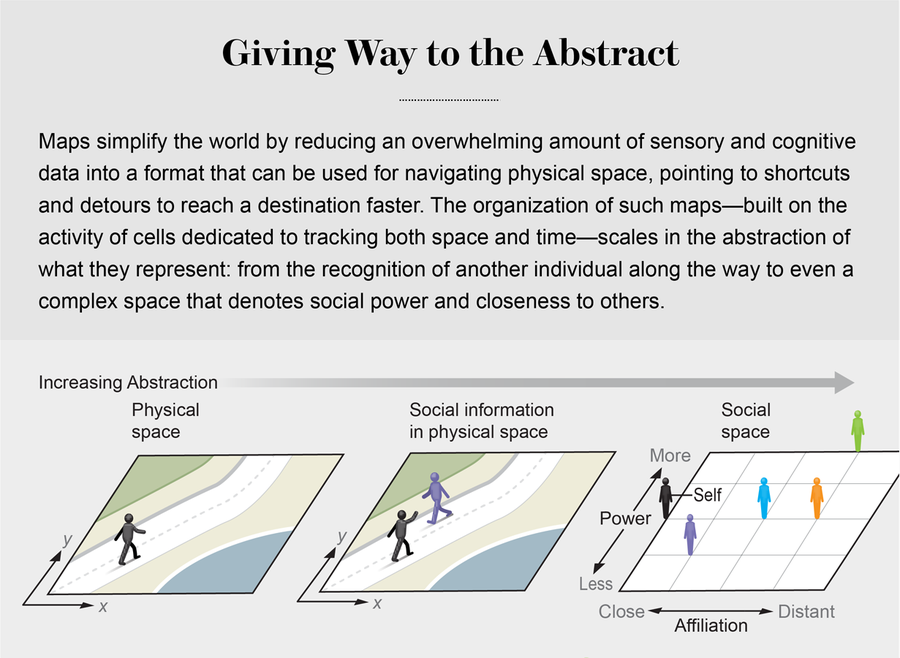
Credit: Jen Christiansen; Source: “Navigating Social Space,” by Matthew Schafer and Daniela Schiller, in Neuron, Vol. 100; October 24, 2018
Mental Time Travel
Time and space are inextricably linked. It is difficult to talk about time without borrowing a spatial metaphor: time “passes” as we “move” through it. We look “forward” to the future and “back” on our past. The same hippocampal-entorhinal system tracks movement through time. Work done largely in the lab of the late Howard Eichenbaum of Boston University revealed neurons in the hippocampal-entorhinal system that encode the time course of an animal's experience. Time cells fire at successive moments but do not track time in a simple clocklike fashion. Instead they mark temporal context—stretching or shrinking their firing durations if the length of a task changes, for example. Some time cells encode space as well. In the brain, in fact, physical and temporal space may be bound together.
The discovery of the crucial importance of these brain areas in space and time was not totally surprising. Psychologists had long suspected it to be the case. In 1953 Henry Molaison underwent bilateral hippocampal resection surgery to reduce extreme, life-disrupting epileptic seizures. The surgery was successful at quelling the seizures. But Molaison—known for decades only as H.M.—became one of the most renowned cases in the history of the brain sciences.
Molaison could remember most experiences from before his surgery—people he knew and recollections from culture and politics. But his ability to form such explicit memories postsurgery was practically nonexistent. Even so, certain types of learning and memory remained untouched: he could still learn some new skills with enough practice. But his recollections of new people, facts and events were immediately lost.
From observing Molaison, neuroscientists discerned that the hippocampus was essential in forming the episodic memories that record facts and events. Research on the role of the hippocampus in episodic memory exploded, largely in parallel to studies on its maplike functions.
The discoveries about the roles of the hippocampus and entorhinal cortex in spatial navigation and episodic memory were significant for at least a couple of reasons. The work in spatial navigation in rodents marked the first time that a higher-order cognitive function—something beyond basic sensory processes—mapped onto clear neural correlates. H.M. showed us that there were multiple types of memory supported by at least partially different neural systems, with the hippocampus playing a central role in the formation and storage of new episodic memories. These discoveries hinted that mechanisms of spatial and temporal navigation might underlie episodic memory. This synthesis is perhaps best explained by the theoretical construct proposed decades earlier by Tolman; both episodic memory and spatial navigation might reflect the brain's formation and use of cognitive maps.
Maps are not accurate portraits of the world in all of its complexity. Rather they are representations of relations—distances and directions between locations and what exists where. Maps reduce a dizzying amount of real-world information into a simple, easily readable format that is useful for effective, flexible navigation. The cell types mentioned earlier (place cells, grid cells and border cells, among others) may piece together such related elements into a mental map, which other brain regions can then read out to guide “navigation,” amounting to adaptive decision-making. Mapping allows relations to be inferred, even when they have not been experienced. It also allows for mental shortcuts that go beyond the purview of the spatial and temporal domains. In fact, reasoning using abstract concepts may depend on some of these same neural foundations.
In one example of this line of work, researchers Alexandra Constantinescu, Jill O'Reilly and Timothy Behrens, all then at the University of Oxford, asked participants to learn associations of different symbols with images of “stick” birds with various neck and leg lengths. A bird with a long neck but short legs, for example, might be linked with the image of a bell, whereas a bird with a short neck and long legs might be connected to a teddy bear. These linkages created a two-dimensional association space. Despite neuroimaging being too crude to detect actual grid cells in the human brain, imaging conducted during the learned- association testing nonetheless revealed a gridlike pattern of activation within the entorhinal cortex.
This finding builds on earlier work by Christian Doeller of the Max Planck Institute for Human Cognitive and Brain Science in Leipzig, Germany, and Neil Burgess of University College London that first showed an entorhinal gridlike representation in humans navigating a virtual maze. For both physical and abstract relations, the gridlike organization is highly efficient. It makes the linkages of places or concepts more predictable, enhancing how quickly inferences can be made about these relations. As in physical space, this organization of information allows for the inference of shortcuts—relations between ideas or perhaps analogies, stereotypes and even some aspects of creativity itself could depend on such inferences.
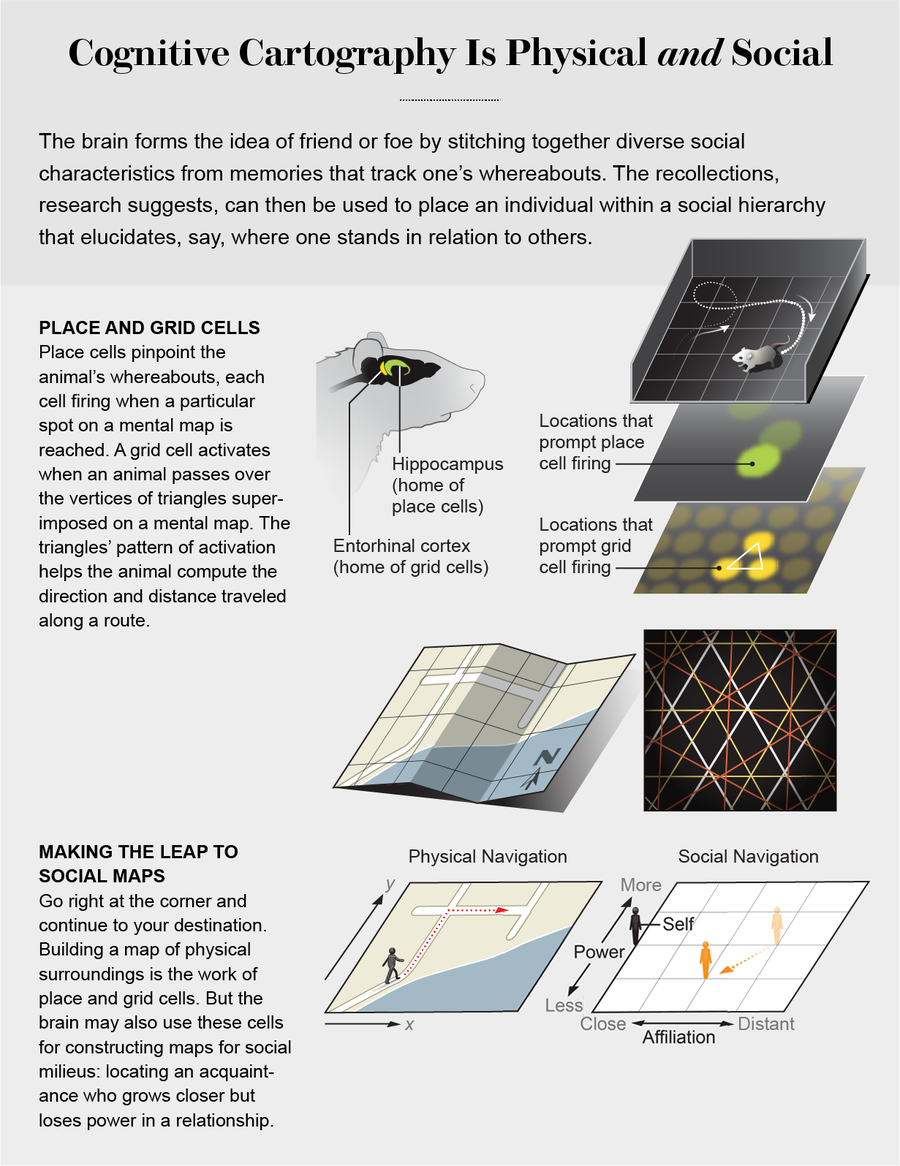
Credit: Jen Christiansen; Sources: “Scientific Background: The Brain’s Navigational Place and Grid Cell System,” by Ole Kiehn and Hans Forssberg, with illustrations by Mattias Karlen. Nobelprize.org; “Navigating Social Space,” by Matthew Schafer and Daniela Schiller, in Neuron, Vol. 100; October 24, 2018
People Maps
The progression from the physical to the abstract carries over into the way the brain represents social relationships. Various bits of knowledge about another person are distilled into the concept of that individual. When we see a photograph of someone or hear or see that person's name, the same hippocampal cells will fire, regardless of the sensory details of the stimulus (for example, the famous “Jennifer Aniston neuron” described by Itzhak Fried of the University of California, Los Angeles, and his colleagues). These hippocampal cells are responsible for representing concepts of specific individuals.
Other hippocampal cells track the physical locations of others and are called social place cells. In an experiment by David Omer of the Hebrew University of Jerusalem, Nachum Ulanovsky of the Weizmann Institute of Science in Rehovot, Israel, and their colleagues, bats observed other bats navigating a simple maze to reach a reward. The task of an observer bat was to simply watch and learn from a navigating bat, enabling it to subsequently navigate the same route to get the same reward. When the observer bat watched, hippocampal cells fired corresponding to the location of the other bat.
Neural circuitry within specific subregions of the hippocampus (in particular, areas called CA1 and CA2) contributes to such social memories. Artificial stimulation or inactivation of these hippocampal areas enhances or diminishes an animal's ability to recognize other animals. In humans, hippocampal injury often spares memory for specific, individual faces, but the relation between this cardinal identifier of another person and that individual's behavior may be lost. That observation suggests that the hippocampus does not simply record a face or some other personal detail but rather ties together diverse social characteristics.
Hippocampal activity also tracks social hierarchies: the demands of a boss and a co-worker, for instance, may be valued differently and confer different social standings. Common metaphors illustrate the spatial dimensions of a hierarchy: a person may try to gain status to “climb the social ladder” or “look down” at someone below them. Other factors are also critical. Biological relatedness, common group goals, the remembered history of favors and slights—all determine social proximity or distance. Human relationships can be conceived of as geometric coordinates in social space that are defined by the dimensions of hierarchy and affiliation.
Work in our lab has explored these ideas in recent years. Our results suggest that, as with other spaces, the hippocampus organizes social information into a maplike format. To test this hypothesis, we put individuals in a choose-your-own-adventure game in which they interacted with cartoon characters and made decisions while their brains were scanned.
In the game, players had just moved to a new town and needed to interact with the fictional characters to secure a job and a place to stay. Participants made decisions on how to deal with a given character. Players could request that others perform favors to demonstrate their power, or they could submit to demands made on them. In a subsequent interaction, they could decide whether to make a gesture of attachment—giving a hug or remaining at a distance.
Using these decisions, we plotted each character at certain coordinates on a map representing their movement along the dimensions of power and affiliation. In each interaction, we drew a line or vector from the participant to the character. In this way, we charted the evolving relations as trajectories through social space and computed information about the angles and lengths of the social vectors.
We searched for neural signals that tracked this information by correlating a participant's brain activity with the angle and length of the vectors for each decision. Activity in the hippocampus tracked the angle of the characters to the participant. The degree to which hippocampal activity captured these social coordinates also reflected the participants' self-reported social skills. These findings suggest that the hippocampus monitors social dynamics as it does physical locations by encoding relations between points in multidimensional space. Indeed, it may be that along any arbitrary dimension in which we can order information, whether physical or abstract, the hippocampus-entorhinal system plays a part.
Many questions about the brain's social maps still remain unanswered. How does this system interact with other brain regions? For example, in our role-playing study, we found that the posterior cingulate cortex, a region also involved in representing spatial information, tracked the length of social vectors—functioning in effect as a measuring stick of “social distance.” Further, a gridlike signal was found in brain regions that are interconnected with and tend to co-activate with the hippocampal-entorhinal system, suggesting they form a network of brain regions with common functional properties.
As research accumulates, questions of clinical importance arise as well. Can flawed mapping processes explain psychiatric dysfunction? Another possibility is that insights garnered from this brain architecture could inform artificial-intelligence development. Well-organized internal models of the world might be key to building more intelligent machines.
That the same mapping system may underlie navigation through space and time, reasoning, memory, imagination and even social dynamics suggests that our ability to construct models of the world might be what makes us such adaptive learners. The world is full of both physical and abstract relations. Road maps of city streets and mental maps of interrelated concepts help us make sense of the world by extracting, organizing and storing related information. A new coffee shop on a familiar street can be easily placed within an existing spatial map. Fresh concepts can be related to older ideas. And a new acquaintance can reshape our social space.
Maps let us simulate possibilities and make predictions, all within the safety of our own heads. The mental shortcuts we can so readily conjure up might have their basis in the same system that allows us to figure out a detour around a traffic jam. We have just begun to discover the varied properties and capacities of this system. Mental maps do more than help us find shortcuts through physical space—they enable us to navigate life itself.”